From SICP section
2.2.3 Sequences as Conventional InterfacesExercise 2.36 asks us to complete the
accumulate-n
procedure, which is similar to
accumulate
except that it takes as its third argument a sequence of sequences that are assumed to all have the same length. It applies the accumulation procedure to all the first elements of the sub-sequences, all the second elements, third elements, and so on and returns a sequence of the results. For example, given the sequence s containing the following values,
((1 2 3) (4 5 6) (7 8 9) (10 11 12))
, the value of
(accumulate-n + 0 s)
should return the sequence
(22 26 30)
.
Our task is to fill in the missing expressions in the following definition:
(define (accumulate-n op init seqs)
(if (null? (car seqs))
null
(cons (accumulate op init <??>)
(accumulate-n op init <??>))))
If you've been doing all the exercises up to this point, then this procedure's overall structure of recursively building up a sequence with
cons
will be familiar.
In
exercise 2.35 we used
map
and a simple lambda expression to flatten out a tree so the result could be used as the third parameter to
accumulate
. We can do something very similar here to fill in the missing expressions above. We'll still use
map
to loop over each of the sub-sequences, but in this case we need
map
to return a sequence that contains the first element from each sub-sequence (in the first missing expression) and the remaining elements of each sub-sequence (in the second missing expression). We already know two procedures that meet those needs,
car
and
cdr
.
; accumulate from sicp section 2.2.3
(define (accumulate op initial sequence)
(if (null? sequence)
initial
(op (car sequence)
(accumulate op initial (cdr sequence)))))
(define (accumulate-n op init seqs)
(if (null? (car seqs))
null
(cons (accumulate op init (map car seqs))
(accumulate-n op init (map cdr seqs)))))
The first call to
map
returns a sequence containing the first element of each of the original sub-sequences. The second call to
map
returns the sequence of sub-sequences with each of their first elements removed. This continues recursively until no more elements remain. We can test the solution with the values given in the exercise.
> (define s (list (list 1 2 3) (list 4 5 6) (list 7 8 9) (list 10 11 12)))
> s
((1 2 3) (4 5 6) (7 8 9) (10 11 12))
> (accumulate-n + 0 s)
(22 26 30)
Exercise 2.37 introduces a representation for vectors and matrices. A vector can be represented as a sequence of numbers, and a
matrix as a sequence of vectors. For example, the matrix
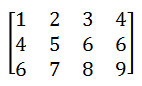
can be represented as the sequence
((1 2 3 4) (4 5 6 6) (6 7 8 9))
.
We can use this representation to express the basic operations of matrix algebra in terms of sequence operations. These basic operations are:
(dot-product v w)
- returns the sum Σ
i(v
iw
i)
(matrix-*-vector m v)
- returns the vector t, where t
i = Σ
j(m
ijv
j)
(matrix-*-matrix m n)
- returns the matrix p, where p
ij = Σ
k(m
ikn
kj)
(transpose mat)
- returns the matrix n, where n
ij = m
jiDot productThe
dot product is defined for us.
(define (dot-product v w)
(accumulate + 0 (map * v w)))
The
dot-product
procedure takes two vectors (that are assumed to be equal length) and returns a single number obtained by multiplying corresponding elements and then summing those products.
Note that the use of
map
in this procedure takes more arguments than we've seen up to this point. That's because
map
takes a procedure of
n arguments, together with
n lists, and applies the procedure to all the first elements of the lists, all the second elements of the lists, and so on, returning a list of the results. Up to this point, we've been using
map
where
n = 1.
Our task is to fill in the missing expressions in the given procedures for computing the remaining matrix operations. Let's take them one at a time.
Multiply a matrix by a vector(define (matrix-*-vector m v)
(map <??> m))
When
multiplying a matrix by a vector, each row of the matrix is multiplied by the vector (using the dot product procedure described above). The result is a vector containing each dot product. Since
map
will pass each row of the matrix to whatever function we provide, we can just define a lambda expression that uses the
dot-product
procedure already defined.
(define (matrix-*-vector m v)
(map (lambda (row) (dot-product row v)) m))
Transpose a matrix(define (transpose mat)
(accumulate-n <??> <??> mat))
There are several ways of looking at
matrix transposition, but the one that makes the most sense given the code above is to write the columns of the input matrix as the rows of the output matrix. Remember that
accumulate-n
will combine the columns with whatever function we give it, and this part of the exercise is a snap.
(define (transpose mat)
(accumulate-n cons null mat))
We can test this procedure with the matrix s defined in the exercise above.
> (define s (list (list 1 2 3) (list 4 5 6) (list 7 8 9) (list 10 11 12)))
> (transpose s)
((1 4 7 10) (2 5 8 11) (3 6 9 12))
Multiply two matrices(define (matrix-*-matrix m n)
(let ((cols (transpose n)))
(map <??> m)))
When
multiplying two matrices m and
n, the resulting matrix will have the same number of rows as
m and the same number of columns as
n. Each element of the result matrix can be found by taking the dot product of each row of
m and each column of
n.
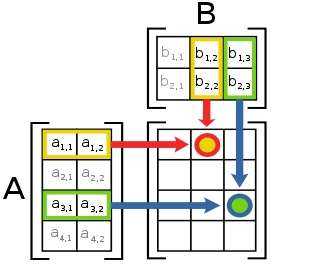
When viewed this way, it's easy to see that each row the resulting matrix is the product of a row in the first input matrix (
m) and the entire second input matrix (
n). Using this knowledge, we can define multiplication of two matrices in terms of
matrix-*-vector
.
(define (matrix-*-matrix m n)
(let ((cols (transpose n)))
(map (lambda (row) (matrix-*-vector cols row)) m)))
TestingWe have to remember a few rules of matrix algebra that aren't enforced in the procedures we've defined.
- For
dot-product
, two vectors can only be multiplied if they're the same length. - For
matrix-*-vector
, the vector must be the same length as the number of columns in the matrix. - For
matrix-*-matrix
, two matrices A and B can be multiplied only if the number of columns of A matches the number of rows of B.
> (define v (list 1 3 -5))
> (define w (list 4 -2 -1))
> (dot-product v w)
3
> (define m (list (list 1 2 3) (list 4 5 6)))
> (define v (list 1 2 3))
> (matrix-*-vector m v)
(14 32)
> (define a (list (list 14 9 3) (list 2 11 15) (list 0 12 17) (list 5 2 3)))
> (define b (list (list 12 25) (list 9 10) (list 8 5)))
> (matrix-*-matrix a b)
((273 455) (243 235) (244 205) (102 160))
Note that the values above are the same as those used as as examples on pages I linked to explaining each operation. The results above are correct, but you can do a Google search for "
matrix multiplication calculator" to find several online calculators if you want to test with other values.
Related:For links to all of the SICP lecture notes and exercises that I've done so far, see
The SICP Challenge.